I’m not a big fan of insects. They have entirely too many legs for me to be comfortable with. Granted, there are exceptions. I like butterflies, big fuzzy bumblebees, dragonflies and grasshoppers. I don’t even mind certain kinds of ants (though not if they’re in my home). Oh, and of course I like the Katydid. But there are some bugs that I just cannot stand. Spiders, earwigs, centipedes, beetles, flies, mosquitoes, wasps, silverfish, termites, cockroaches, mites, ticks, weevils, scorpions, fleas… you get the idea. They make my skin crawl. Of course, I know it’s somewhat irrational. Even though some insects are poisonous, most have more reason to be scared of me than I do of them. And I’m easily many orders of magnitude larger than them – so it’s easy enough for me to squish them.
It’s this size difference that I want to talk about today – specifically in light of an article that I found which made me realize that bugs were not always the size they are today.
Paleontologists from the University of Bristol have recently discovered a fossil of the world’s largest bug – at a whopping 2.5 meters long. That’s 8 feet. That’s over 2 feet longer than I am tall! Whoa.
(To see a diagram comparing this insect to a human, you can see one at: http://www.msnbc.msn.com/id/21906979/)
The bug in question is an Eurypterid, or ancient sea scorpion. Eurypterids are believed to be aquatic ancestors of modern-day arachnids (which includes scorpions, spiders, and horseshoe crabs). They were predators of the ancient seas, from the Cambrian to the Permian periods (500 to 250 million years ago). Eurypterids had segmented bodies, a long, tapering, flexible tail, and claws protruding from their heads. They were ubiquitous in their day, and their fossils have been discovered all around the world. Most of the fossils are of small specimens – 1 to 15 inches long. However, the fossil announced this year was 18 inches long – and that was just one of its claws!
The finding was just published in the November edition of the Royal Society's Biology letters. However, the fossil itself was discovered several years ago in Germany by paleobiologist Dr. Markus Poschmann. Since then, Dr. Poschmann has worked with Dr. Simon Braddy to determine how big an animal would have to be to have a claw of that size. It turns out that sea scorpions have a constant ratio between claw size and body length. With that ratio, they calculated that a sea scorpion with an 18 inch claw would have a body 8 feet long – 11 feet if you include the reach of the claws.
Exactly how and why this creature grew so large is unknown. Scientists have long known that giant land-based bugs existed during the Carboniferous period, around 300 million years ago. At that time, there was a boost in atmospheric oxygen, which might have allowed creatures that use breathing systems that diffuse oxygen into tissues (like insects) to get bigger and bigger. However, two things argue against this being the reason for the giant sea scorpion. First, it predates this increase in atmospheric oxygen. And second, this beast was too big to come onto land, so it wouldn’t have even used atmospheric oxygen. So it is unlikely that this atmospheric oxygen boost is the cause of this giant bug. Dr. Braddy speculates that eurypterids grew increasingly large to be better able to prey on armoured fish. Moreover, there was not much competition from vertebrates at that time, and the sea scorpions themselves had no predators. So they could get as large as they wanted. However, eventually these giant bugs had to downsize due to competition from large fish with jaws and teeth. They eventually disappeared from the fossil record during the Permian extinction approximately 250 million years ago, which wiped out 95% of marine species.
Personally, I’m glad that we don’t have insects this large around anymore. If I get the creepy-crawlies from a spider less than an inch in diameter, imagine what I’d do if faced with a scorpion 8 feet long! (On second thought, let's not imagine that. It's too creepy.)
Tuesday, November 27, 2007
Monday, November 26, 2007
Mirror Images and Molecules
In my last entry on turkey, I mentioned that the proper name for the amino acid tryptophan is really L-tryptophan. Though we usually leave the L out, it is actually very important, at least as far as the chemistry is concerned. The L designates a characteristic of the molecule that is called chirality (pronounced k-EYE-ral-it-ee).
Chirality comes from the Greek word meaning “hand,” and is a property of asymmetry. To understand chirality, take a look at your hands. To a certain extent, your hands are identical – 4 fingers and 1 thumb, all of comparable sizes and arranged the same way around palms of equal size. However, your hands are different in one important respect – you cannot overlay them. If you put your right and left hands in the same orientation (say, palm down), and then put your right hand on top of your left, they don’t match. Your thumbs are on opposite sides. That’s because your hands are really mirror images of each other. Hold them palm-to-palm, and they match up.
An object is chiral if it cannot be superimposed on its mirror image. I’ve given you an example in the diagram below. There are a few important notes that you need to know to understand a picture like this. First, it is a 2-dimensional image of a 3-dimensional object, so you’ll have to use your imagination a little. Second, each letter represents a different atom. Third, the lines connecting the atoms are bonds – chemical links that hold the 2 atoms together. You can’t break the bonds, or shuffle the orientation of the atoms relative to each other. A bond designated by a solid triangle points out of the picture at you, while an empty triangle points into the picture away from you. A single line represents a bond flat in the plane of the picture.
In this diagram, the central atom (N) is bonded to 3 other atoms (X, Y, and Z).
In the diagram on the left, N and X are in the plane of the picture (flat), Y points out of the picture at you, and Z is behind the picture away from you. In the center panel is the mirror image. Its mirror image is the same (N and X in the plane, Y pointing out, and Z pointing back). However, if I now flip the image on the left to try and superimpose back on the original (that's on the right), all of a sudden it doesn’t work. Though N and X are still in the plane, now Z points out at you and Y points back. These molecules have the same chemical composition, the same type and number of atoms, and the bonds connect the atoms in the same way in both. But the 2 molecules are mirror images of each other, and they cannot be overlaid. That makes them chiral.
But who cares if you can superimpose them or not? They’re still the same molecule, right? Actually, no, they’re not. So let’s go back to L-tryptophan. Tryptophan is a chiral molecule, which means that it is not the same as its mirror image. The 2 mirror image versions of tryptophan (called isomers) are L-tryptophan and D-tryptophan. Your body cannot use D-tryptophan at all. It can only use L-tryptophan - as a building block for proteins, as a precursor for serotonin, or for any other metabolic process. In fact, almost all of the amino acids in your body are L. (The exception is glycine, which is so simple a molecule that it doesn’t have chirality.) The only organisms to use D amino acids are some bacteria and a few exotic sea creatures. Even if you were to eat D-amino acids, you can’t use them. Your body would just get rid of them.
I find it fascinating that a molecule - like an amino acid – can be chemically identical to another (as in its mirror image), and yet the 2 have totally different properties in your body. Our body chemistries are really exquisitely specific!
Chirality comes from the Greek word meaning “hand,” and is a property of asymmetry. To understand chirality, take a look at your hands. To a certain extent, your hands are identical – 4 fingers and 1 thumb, all of comparable sizes and arranged the same way around palms of equal size. However, your hands are different in one important respect – you cannot overlay them. If you put your right and left hands in the same orientation (say, palm down), and then put your right hand on top of your left, they don’t match. Your thumbs are on opposite sides. That’s because your hands are really mirror images of each other. Hold them palm-to-palm, and they match up.
An object is chiral if it cannot be superimposed on its mirror image. I’ve given you an example in the diagram below. There are a few important notes that you need to know to understand a picture like this. First, it is a 2-dimensional image of a 3-dimensional object, so you’ll have to use your imagination a little. Second, each letter represents a different atom. Third, the lines connecting the atoms are bonds – chemical links that hold the 2 atoms together. You can’t break the bonds, or shuffle the orientation of the atoms relative to each other. A bond designated by a solid triangle points out of the picture at you, while an empty triangle points into the picture away from you. A single line represents a bond flat in the plane of the picture.
In this diagram, the central atom (N) is bonded to 3 other atoms (X, Y, and Z).
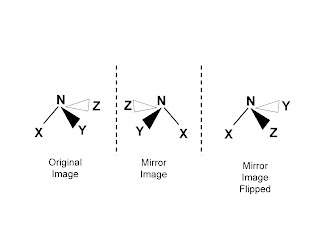
But who cares if you can superimpose them or not? They’re still the same molecule, right? Actually, no, they’re not. So let’s go back to L-tryptophan. Tryptophan is a chiral molecule, which means that it is not the same as its mirror image. The 2 mirror image versions of tryptophan (called isomers) are L-tryptophan and D-tryptophan. Your body cannot use D-tryptophan at all. It can only use L-tryptophan - as a building block for proteins, as a precursor for serotonin, or for any other metabolic process. In fact, almost all of the amino acids in your body are L. (The exception is glycine, which is so simple a molecule that it doesn’t have chirality.) The only organisms to use D amino acids are some bacteria and a few exotic sea creatures. Even if you were to eat D-amino acids, you can’t use them. Your body would just get rid of them.
I find it fascinating that a molecule - like an amino acid – can be chemically identical to another (as in its mirror image), and yet the 2 have totally different properties in your body. Our body chemistries are really exquisitely specific!
Tuesday, November 20, 2007
Don’t blame the turkey!
In honor of the upcoming Thanksgiving holiday, I wanted to write about a scientific topic that I’m sure will be brought up in many households across the country. Sometime after dinner, you may find yourself sitting on the couch, trying to stay awake. And you just might think:
The tryptophan in the turkey I just ate is making me sleepy.
A Newsweek article that I came across today definitely bolsters this idea: “Four Reasons Thanksgiving Makes Us Sleepy.” Reason #1? The turkey and the trimmings.
But is it true? Does eating a tryptophan-rich food like turkey really make you sleepy? If yes, why? If not, why does everyone think it does? For that matter, what is tryptophan, anyways?
Tryptophan is an amino acid, one of 20 standard amino acids used as building blocks to make proteins. (Actually, its proper name is really L-tryptophan, but hardly anybody uses the L.) Tryptophan is a pretty complex amino acid, considered hydrophobic (which means it doesn’t like water) and aromatic (which refers to its chemical structure). It is actually one of the essential amino acids, which means that our bodies cannot make it ourselves. We have to get it from our diet. (Nine of the twenty standard amino acids are essential; we can produce the other eleven.) Tryptophan is found from several food sources, including poultry (both chicken and turkey), pork, cheese, beef, fish, peanuts and soybeans. (Basically, foods rich in protein are also rich in tryptophan.) In addition to being used as a building block for proteins, tryptophan is also used by our bodies to make hormones. For example, tryptophan is used to make niacin (a B-vitamin). Niacin, in turn, is used to make serotonin. And serotonin is a remarkable hormone that exerts a calming effect on your brain, and plays a key role in making you sleepy. Tryptophan is also used in the production of melatonin, another hormone that regulates sleep.
With all that, it makes sense to think that eating a tryptophan-rich meal would result in increased levels of serotonin in your brain, which would then make you drowsy, right? In fact, in the 1980s, many people took L-tryptophan supplements to help combat insomnia. It’s not taken in the US anymore, as the FDA has banned it due to problems with production. But L-tryptophan supplements are still used to treat insomnia in Canada – though it’s only available by prescription.
Unfortunately, while the idea sounds good in theory, it doesn’t really work that way. In order for tryptophan to cause sleepiness, it must get to the brain. To do that, it must be eaten on an empty stomach, with no other protein source. If eaten with other proteins, all of the amino acids are trying to go to the brain at once. And since they all use the same transport system to get there, too many amino acids at once make it slower for all of them. (Just like driving during rush hour.)
So why do we get sleepy after Thanksgiving dinner? One of the biggest factors is that we simply eat a lot. To digest food, your body diverts blood away from your brain and down to your digestive system. The more food that has to be digested, the more blood has to be diverted to your stomach. This is especially true if you ingest a lot of fats in the meal. Fats (for example, in the meat, gravy, butter and desserts) take a longer time to digest than other components of your meal. So not only does your body need to use a lot of blood to digest the quantity of food, it needs extra time to digest the quality of food. And all that blood diverted from your brain to your stomach means sleepiness.
Another big food culprit is the carbohydrates (found in potatoes, bread, yams, stuffing and dessert). A carbohydrate-rich meal causes your pancreas to secrete insulin. When the insulin hits your blood stream, it causes your muscle cells to absorb glucose out of the blood. Since glucose is essentially energy, reducing your blood glucose level results in a feeling of low energy. Have you ever eaten a lot of sugar? You probably got a big boost of energy that quickly wore away, leaving you feeling very tired. That’s because sugar causes a rise in blood glucose, which gives you energy. But your body compensates by producing insulin, which pulls that glucose out of your bloodstream, leaving you tired.
I do want to address an issue raised in the Newsweek article I mentioned above. This article cites research published in the Canadian Journal of Physiology and Pharmacology in 2007. The research report, entitled “Protein-source tryptophan as an efficacious treatment for social anxiety disorder: a pilot study,” argues that tryptophan reaches the brain better when eaten in combination with carbohydrates than when eaten by itself. That’s because insulin causes specific absorption of some amino acids into your muscle cells, but not tryptophan. These other amino acids can no longer compete with tryptophan for delivery to the brain. The authors of the research say that serotonin increases in the brain, as measured by an anxiety test. (Remember, serotonin helps calm you down.) Two big problems with this study, though are (a) they didn’t test sleepiness, and (b) they tested pure components – deoiled gourd seed (a tryptophan source) and pure glucose (a carbohydrate). So it’s a stretch to say that this proves that turkey makes you sleepy on Thanksgiving. Relative to the other 2 factors mentioned above (blood diversion and insulin production), sleepiness induced by serotonin in your brain from ingested tryptophan is going to be small. You’re going to fall asleep because of the amount of food you ate much sooner than you would from the tryptophan in your turkey.
So while you may feel sleepy after dinner on Thursday, don’t blame the turkey. It gets a bad reputation for keeping us prone on the couch, when we should probably be helping with the dishes!
The tryptophan in the turkey I just ate is making me sleepy.
A Newsweek article that I came across today definitely bolsters this idea: “Four Reasons Thanksgiving Makes Us Sleepy.” Reason #1? The turkey and the trimmings.
But is it true? Does eating a tryptophan-rich food like turkey really make you sleepy? If yes, why? If not, why does everyone think it does? For that matter, what is tryptophan, anyways?
Tryptophan is an amino acid, one of 20 standard amino acids used as building blocks to make proteins. (Actually, its proper name is really L-tryptophan, but hardly anybody uses the L.) Tryptophan is a pretty complex amino acid, considered hydrophobic (which means it doesn’t like water) and aromatic (which refers to its chemical structure). It is actually one of the essential amino acids, which means that our bodies cannot make it ourselves. We have to get it from our diet. (Nine of the twenty standard amino acids are essential; we can produce the other eleven.) Tryptophan is found from several food sources, including poultry (both chicken and turkey), pork, cheese, beef, fish, peanuts and soybeans. (Basically, foods rich in protein are also rich in tryptophan.) In addition to being used as a building block for proteins, tryptophan is also used by our bodies to make hormones. For example, tryptophan is used to make niacin (a B-vitamin). Niacin, in turn, is used to make serotonin. And serotonin is a remarkable hormone that exerts a calming effect on your brain, and plays a key role in making you sleepy. Tryptophan is also used in the production of melatonin, another hormone that regulates sleep.
With all that, it makes sense to think that eating a tryptophan-rich meal would result in increased levels of serotonin in your brain, which would then make you drowsy, right? In fact, in the 1980s, many people took L-tryptophan supplements to help combat insomnia. It’s not taken in the US anymore, as the FDA has banned it due to problems with production. But L-tryptophan supplements are still used to treat insomnia in Canada – though it’s only available by prescription.
Unfortunately, while the idea sounds good in theory, it doesn’t really work that way. In order for tryptophan to cause sleepiness, it must get to the brain. To do that, it must be eaten on an empty stomach, with no other protein source. If eaten with other proteins, all of the amino acids are trying to go to the brain at once. And since they all use the same transport system to get there, too many amino acids at once make it slower for all of them. (Just like driving during rush hour.)
So why do we get sleepy after Thanksgiving dinner? One of the biggest factors is that we simply eat a lot. To digest food, your body diverts blood away from your brain and down to your digestive system. The more food that has to be digested, the more blood has to be diverted to your stomach. This is especially true if you ingest a lot of fats in the meal. Fats (for example, in the meat, gravy, butter and desserts) take a longer time to digest than other components of your meal. So not only does your body need to use a lot of blood to digest the quantity of food, it needs extra time to digest the quality of food. And all that blood diverted from your brain to your stomach means sleepiness.
Another big food culprit is the carbohydrates (found in potatoes, bread, yams, stuffing and dessert). A carbohydrate-rich meal causes your pancreas to secrete insulin. When the insulin hits your blood stream, it causes your muscle cells to absorb glucose out of the blood. Since glucose is essentially energy, reducing your blood glucose level results in a feeling of low energy. Have you ever eaten a lot of sugar? You probably got a big boost of energy that quickly wore away, leaving you feeling very tired. That’s because sugar causes a rise in blood glucose, which gives you energy. But your body compensates by producing insulin, which pulls that glucose out of your bloodstream, leaving you tired.
I do want to address an issue raised in the Newsweek article I mentioned above. This article cites research published in the Canadian Journal of Physiology and Pharmacology in 2007. The research report, entitled “Protein-source tryptophan as an efficacious treatment for social anxiety disorder: a pilot study,” argues that tryptophan reaches the brain better when eaten in combination with carbohydrates than when eaten by itself. That’s because insulin causes specific absorption of some amino acids into your muscle cells, but not tryptophan. These other amino acids can no longer compete with tryptophan for delivery to the brain. The authors of the research say that serotonin increases in the brain, as measured by an anxiety test. (Remember, serotonin helps calm you down.) Two big problems with this study, though are (a) they didn’t test sleepiness, and (b) they tested pure components – deoiled gourd seed (a tryptophan source) and pure glucose (a carbohydrate). So it’s a stretch to say that this proves that turkey makes you sleepy on Thanksgiving. Relative to the other 2 factors mentioned above (blood diversion and insulin production), sleepiness induced by serotonin in your brain from ingested tryptophan is going to be small. You’re going to fall asleep because of the amount of food you ate much sooner than you would from the tryptophan in your turkey.
So while you may feel sleepy after dinner on Thursday, don’t blame the turkey. It gets a bad reputation for keeping us prone on the couch, when we should probably be helping with the dishes!
Friday, November 16, 2007
Language and Sound – A Baby’s Sophisticated Brain
I recently had the pleasure of listening to a seminar entitled “Learning Language,” which was about many different aspects of how babies and children acquire language skills. It was given by Dr. Patricia Kuhl, Professor of Speech and Hearing Sciences at the University of Washington’s Institute for Brain and Learning Sciences. Dr. Kuhl is internationally renowned for her research on brain development and language acquisition in infants and young children, and is one of the leading experts in the world. This was one of the more interesting seminars I’ve ever sat through – and believe me, I’ve sat through many seminars during my years as a scientist! Before this seminar, I had never really thought about the science behind how we learn language. But now that I know a little bit about it, it’s a fascinating topic.
To begin, let me tell you a story. I had a Chinese-American roommate fluent in both English and Mandarin. She once told me a popular Chinese children’s rhyme, which was in Mandarin. It was the strangest sounding children’s rhyme I’d ever heard! To me, it sounded like the same word, repeated over and over. To her, however, the words were different – distinguished by tonal differences in each word that her ears could detect. (If you’ve ever had experience with Asian languages, this may sound familiar to you!) The reverse is also true. Native Japanese speakers, for example, often cannot hear sound differences in the English language, such as the difference between “ra” and “la.”
Have you ever wondered why this is? I mean, it’s just sounds, right? I can hear pretty well – so why can’t I hear the tonal differences associated with Mandarin Chinese? Why can’t a native Japanese speaker distinguish an “L” from an “R?” It’s not just that we can’t pronounce them right – we can’t even hear them.
One possible answer is something called the “Native Language Magnet/Neural Commitment Theory.” Babies are born with the ability to recognize a vast range of differences in sounds, and studies have shown that they can distinguish differences in sounds from languages all over the world. However, once they’ve reached a year of age, their ability to distinguish sounds from foreign languages is greatly diminished. An example showed by Dr. Kuhl concerns the ability of Japanese and American babies to distinguish “ra” from “la.” Both sets of babies can distinguish “ra” from “la” very efficiently at 6-8 months of age. However, at 12 months, Japanese babies are only half as likely the be able to hear the difference as American babies; their discernment gets worse as they get older.
According to the Native Language Magnet/Neural Commitment Theory, the brains of babies are constantly listening to the world around them. And the sounds that they hear most often are the ones that their brains hone in on. An American baby, for example, will hear “ra” and “la” quite often in the speech of those around her. So her brain recognizes that the difference must be important! Therefore, it puts special effort into remembering the difference between the sounds. A Japanese baby, on the other hand, will likely never hear the sound “ra” - it doesn’t exist in the Japanese language. His brain will therefore have no reason to make an effort to remember the difference between the sounds. Instead, his brain will focus on important tonal sounds in the Japanese language.
In this theory, the infant brain passes through 4 phases. Phase 1 is the initial state, in which infants can discern phonetic differences of a wide variety. Phase 2 is the neural commitment state. Auditory processing results in the classification of vowels and consonants into “bins,” or distinguishable groups. When 2 different sounds are put into the same bin, the brain loses the ability to distinguish between them. A Japanese infant will group “la” and “ra” in the same bin – and thus, to them, it becomes the same sound. Phase 3 is really an enhancement of phase 2, in which these phonetic groupings get reinforced. And in phase 4, this neural commitment is stable. The infant brain has now developed in such a way that it can distinguish between the sounds of its native language, but not of other languages.
One interesting ramification of this work is that the sounds a baby hears is really important! He may not know the words you use, but he can use the sounds you make to build his foundation for understanding the language as a whole. It’s the sounds that matter. This means that baby talk is actually really, really important.
In baby talk (also known as “mother-ese” or “infant directed language”), adults exaggerate the phonetic sounds they make. The vowels are stretched, the pitch changes are exaggerated, and the words are slowed down. The end result is to make the sounds even easier for babies to hear and distinguish. Babies find this kind of talk immensely appealing – studies have repeatedly shown that baby talk holds an infant’s attention better and longer than does regular speech. And in terms of the neural commitment theory, baby talk makes it clear to infants what sounds are important for their native language. So evidence indicates that baby talk actually helps infants in the early stages of language acquisition.
There are vast reams of research on language acquisition and skills that I haven’t even hinted at here. It’s an immensely complicated subject, studied by neurobiologists, behavioral psychologists, and linguists, to name a few. But once again, I am amazed at how well our brains handle such an enormously complex thing – especially when we’re as young as 6 months old.
To begin, let me tell you a story. I had a Chinese-American roommate fluent in both English and Mandarin. She once told me a popular Chinese children’s rhyme, which was in Mandarin. It was the strangest sounding children’s rhyme I’d ever heard! To me, it sounded like the same word, repeated over and over. To her, however, the words were different – distinguished by tonal differences in each word that her ears could detect. (If you’ve ever had experience with Asian languages, this may sound familiar to you!) The reverse is also true. Native Japanese speakers, for example, often cannot hear sound differences in the English language, such as the difference between “ra” and “la.”
Have you ever wondered why this is? I mean, it’s just sounds, right? I can hear pretty well – so why can’t I hear the tonal differences associated with Mandarin Chinese? Why can’t a native Japanese speaker distinguish an “L” from an “R?” It’s not just that we can’t pronounce them right – we can’t even hear them.
One possible answer is something called the “Native Language Magnet/Neural Commitment Theory.” Babies are born with the ability to recognize a vast range of differences in sounds, and studies have shown that they can distinguish differences in sounds from languages all over the world. However, once they’ve reached a year of age, their ability to distinguish sounds from foreign languages is greatly diminished. An example showed by Dr. Kuhl concerns the ability of Japanese and American babies to distinguish “ra” from “la.” Both sets of babies can distinguish “ra” from “la” very efficiently at 6-8 months of age. However, at 12 months, Japanese babies are only half as likely the be able to hear the difference as American babies; their discernment gets worse as they get older.
According to the Native Language Magnet/Neural Commitment Theory, the brains of babies are constantly listening to the world around them. And the sounds that they hear most often are the ones that their brains hone in on. An American baby, for example, will hear “ra” and “la” quite often in the speech of those around her. So her brain recognizes that the difference must be important! Therefore, it puts special effort into remembering the difference between the sounds. A Japanese baby, on the other hand, will likely never hear the sound “ra” - it doesn’t exist in the Japanese language. His brain will therefore have no reason to make an effort to remember the difference between the sounds. Instead, his brain will focus on important tonal sounds in the Japanese language.
In this theory, the infant brain passes through 4 phases. Phase 1 is the initial state, in which infants can discern phonetic differences of a wide variety. Phase 2 is the neural commitment state. Auditory processing results in the classification of vowels and consonants into “bins,” or distinguishable groups. When 2 different sounds are put into the same bin, the brain loses the ability to distinguish between them. A Japanese infant will group “la” and “ra” in the same bin – and thus, to them, it becomes the same sound. Phase 3 is really an enhancement of phase 2, in which these phonetic groupings get reinforced. And in phase 4, this neural commitment is stable. The infant brain has now developed in such a way that it can distinguish between the sounds of its native language, but not of other languages.
One interesting ramification of this work is that the sounds a baby hears is really important! He may not know the words you use, but he can use the sounds you make to build his foundation for understanding the language as a whole. It’s the sounds that matter. This means that baby talk is actually really, really important.
In baby talk (also known as “mother-ese” or “infant directed language”), adults exaggerate the phonetic sounds they make. The vowels are stretched, the pitch changes are exaggerated, and the words are slowed down. The end result is to make the sounds even easier for babies to hear and distinguish. Babies find this kind of talk immensely appealing – studies have repeatedly shown that baby talk holds an infant’s attention better and longer than does regular speech. And in terms of the neural commitment theory, baby talk makes it clear to infants what sounds are important for their native language. So evidence indicates that baby talk actually helps infants in the early stages of language acquisition.
There are vast reams of research on language acquisition and skills that I haven’t even hinted at here. It’s an immensely complicated subject, studied by neurobiologists, behavioral psychologists, and linguists, to name a few. But once again, I am amazed at how well our brains handle such an enormously complex thing – especially when we’re as young as 6 months old.
Tuesday, November 13, 2007
Planning for the future
Take a look around you one day and you will see a pattern in human thinking. Each Friday, grocery shoppers take lists to the store to buy food for their families to eat the next week. Each month, paychecks in America are taxed by the Social Security Administration for the money to be returned to those who are retired. Each year, Christmas decorations are put into storage for millions of households to be reused the following holiday season.
Every day of our lives, we plan for the future in some way or another. We save food, money, or supplies. We plan careers, houses and vacations. Do we eat dinner early to watch our favorite TV show, do we hire a babysitter this weekend to go see a movie, or do we really want to go for a jog to reap health benefits years from now? Planning is so common in our lives that it might surprise you to realize that knowing how to plan for the future has always been believed to be an ability exclusive to humans. Animals can exhibit saving behavior. Any city dweller sees how squirrels hide acorns every fall, and dog owners know how their pets bury their bones to be dug up later. However, these behaviors fall short of the true scientific definition of “planning ahead.”
Let’s talk about what qualifies a behavior as “planning ahead.” To non-scientists, planning ahead is a state of mind where you think beyond the present, anticipating a need and doing something ahead of time to fill it. To biologists, however, the definition of planning ahead is more rigorous and based in the animal’s behavior, not their state of mind. After all, we can’t ask squirrels why they bury acorns in the forest.
Two things determine whether an animal is really planning ahead. First, the behavior must be a novel action or actions, something new that the animal has not done before. Second, it must be appropriate to a motivational state, rather than a current condition. In other words, it has to be something the animal will anticipate needing, rather than something it currently needs. Thus a bear fattening up for hibernation cannot be said to be planning ahead for the winter, since it fails both criteria. The bear does it every year after learning from watching their mothers, so it is not a novel action. Moreover, metabolic changes in the bear’s body result in the addition of layers of fat to its anatomy. Biologically speaking, then, it is not a planned response to future hunger but to a current condition of hunger.
Proving whether an animal can plan ahead is very tricky, so it has never been done before. Until recently, that is. Early this year, researchers working with Dr. Nicholas Clayton at the University of Cambridge found a way to test the planning skills of a small, unassuming bird.
Western scrub jays are native to western north America. These birds, which live in oak woodlands in the wild, are well adapted to suburban environments. They are omnivores, and will eat just about anything, including a variety of seed. Importantly for Dr. Clayton, they are known to store their seed. But does that mean that they are planning ahead? Or are they acting on instinct?
Dr. Clayton tested the jays in a variety of caged environments with differing availability of food. In one experiment, the birds were allowed access to three cages. The first evening, the middle cage contained a supply of powdered pine nuts. Jays can’t carry this, thus they can’t save it. The next morning, they were contained for two hours into one of the other two cages, one that contained breakfast and one that did not. After several days of this, the birds were given whole pine nuts for dinner instead of ground pine nuts. The next morning, the scientists discovered a supply of whole pine nuts in the “no breakfast” room, but not the “breakfast” room. The jays knew which cage would not have food in the morning, so at their first chance, they stored food there. All the data for this experiment came from one test, so the birds could not have learned over time how their actions would determine whether or not they would have whole pine nuts to eat the next morning. The conclusion: the jays were planning ahead for their next breakfast.
A second experiment expanded the results. This time, the birds were confined to one of the two side cages for breakfast, where they were given one of two kinds of food. In one room they were fed whole pine nuts, and in the other, they were fed dog kibble. After several mornings of this, they were given both kinds of food in the central room. At this one and only opportunity, the birds stored the type of food that each room was lacking for the next morning (dog kibble in the pine nuts cage and vice versa). Again, the conclusion was clear: this behavior fit the definition of planning ahead.
This story, published earlier this year in the journal Nature, was the first generally accepted scientific proof of a non-human animal planning ahead. And I hope that you get a few things out of this story. First, I think the results are fascinating. Who would have thought that blue jays could think abstractly about the future? But in addition, it really shows how complex living systems are. We can observe a behavior in an animal, but that does not mean we know why they do it. That’s extremely difficult to test. But along with that difficulty comes one of the fun things about being a scientist. We have to be extremely creative sometimes to find a solution to an incredibly complex problem!
Every day of our lives, we plan for the future in some way or another. We save food, money, or supplies. We plan careers, houses and vacations. Do we eat dinner early to watch our favorite TV show, do we hire a babysitter this weekend to go see a movie, or do we really want to go for a jog to reap health benefits years from now? Planning is so common in our lives that it might surprise you to realize that knowing how to plan for the future has always been believed to be an ability exclusive to humans. Animals can exhibit saving behavior. Any city dweller sees how squirrels hide acorns every fall, and dog owners know how their pets bury their bones to be dug up later. However, these behaviors fall short of the true scientific definition of “planning ahead.”
Let’s talk about what qualifies a behavior as “planning ahead.” To non-scientists, planning ahead is a state of mind where you think beyond the present, anticipating a need and doing something ahead of time to fill it. To biologists, however, the definition of planning ahead is more rigorous and based in the animal’s behavior, not their state of mind. After all, we can’t ask squirrels why they bury acorns in the forest.
Two things determine whether an animal is really planning ahead. First, the behavior must be a novel action or actions, something new that the animal has not done before. Second, it must be appropriate to a motivational state, rather than a current condition. In other words, it has to be something the animal will anticipate needing, rather than something it currently needs. Thus a bear fattening up for hibernation cannot be said to be planning ahead for the winter, since it fails both criteria. The bear does it every year after learning from watching their mothers, so it is not a novel action. Moreover, metabolic changes in the bear’s body result in the addition of layers of fat to its anatomy. Biologically speaking, then, it is not a planned response to future hunger but to a current condition of hunger.
Proving whether an animal can plan ahead is very tricky, so it has never been done before. Until recently, that is. Early this year, researchers working with Dr. Nicholas Clayton at the University of Cambridge found a way to test the planning skills of a small, unassuming bird.
Western scrub jays are native to western north America. These birds, which live in oak woodlands in the wild, are well adapted to suburban environments. They are omnivores, and will eat just about anything, including a variety of seed. Importantly for Dr. Clayton, they are known to store their seed. But does that mean that they are planning ahead? Or are they acting on instinct?
Dr. Clayton tested the jays in a variety of caged environments with differing availability of food. In one experiment, the birds were allowed access to three cages. The first evening, the middle cage contained a supply of powdered pine nuts. Jays can’t carry this, thus they can’t save it. The next morning, they were contained for two hours into one of the other two cages, one that contained breakfast and one that did not. After several days of this, the birds were given whole pine nuts for dinner instead of ground pine nuts. The next morning, the scientists discovered a supply of whole pine nuts in the “no breakfast” room, but not the “breakfast” room. The jays knew which cage would not have food in the morning, so at their first chance, they stored food there. All the data for this experiment came from one test, so the birds could not have learned over time how their actions would determine whether or not they would have whole pine nuts to eat the next morning. The conclusion: the jays were planning ahead for their next breakfast.
A second experiment expanded the results. This time, the birds were confined to one of the two side cages for breakfast, where they were given one of two kinds of food. In one room they were fed whole pine nuts, and in the other, they were fed dog kibble. After several mornings of this, they were given both kinds of food in the central room. At this one and only opportunity, the birds stored the type of food that each room was lacking for the next morning (dog kibble in the pine nuts cage and vice versa). Again, the conclusion was clear: this behavior fit the definition of planning ahead.
This story, published earlier this year in the journal Nature, was the first generally accepted scientific proof of a non-human animal planning ahead. And I hope that you get a few things out of this story. First, I think the results are fascinating. Who would have thought that blue jays could think abstractly about the future? But in addition, it really shows how complex living systems are. We can observe a behavior in an animal, but that does not mean we know why they do it. That’s extremely difficult to test. But along with that difficulty comes one of the fun things about being a scientist. We have to be extremely creative sometimes to find a solution to an incredibly complex problem!
Thursday, November 8, 2007
Cats, cats cats!
Today’s topic is one near and dear to my heart – cats. I think cats are among the most fascinating animals on earth. Whether they are domesticated or wild, whether they are big or small, whether they are cute or ferocious, there is something about all of them that I find irresistible.
The list of wild cats is vast and varied. You probably already know about the most famous of the wild cats, such as lions, tigers, cheetahs and leopards. But I thought I’d take some time to tell you about a few wild cats that you might not have heard of before.
For example, take the caracal. The caracal, also known as the African Lynx or the Deser
t Lynx, is found in northern Africa, the Arabian peninsula, and southwestern Asia. They are one of the largest “small” wild cats, and their relatively small bodies are very stocky. Males are about 2 feet long (plus another foot for their tails), and they weigh in at an average of 28-50 pounds. Caracals look somewhat like a cougar - their bodies are usually reddish-brown, and they have white chins, throats, and underbellies. By far their most distinctive feature is their ears. A caracal’s ears are long, tufted and tinged with black. (The name Caracal actually comes from the Turkish word for “black ear” – karakulak.) These ears are one of their most important assets for hunting – each one is controlled by 20 muscles, allowing them to pivot and pinpoint prey. Like all cats, caracals are strict carnivores, subsisting on rodents, birds and small deer. They are nocturnal and highly territorial, which means that they are very difficult to find in the wild. However, they are relatively easy to tame, and there are numerous examples of pet caracals that have been raised from kittenhood by humans.
Another relatively obscure wild cat is the serval. Servals are considered medium-sized wild cats; though their weight is comparable with the caracal (20-45 pounds), their bodies are significantly longer (3 feet in length, plus a foot and a half for the tail). This makes them very slender animals, an image assisted by the fact that they have one of the longest legs in the cat family. (
That, though, is due to really long feet rather than legs). Like leopards, servals have tawny-gold bodies marked with round, black spots. In general, these spots tend to be large, and merge into stripes on their necks and backs. Servals are excellent hunters, especially of rodents. Small mammals, in fact, make up approximately 90% of their diets. This includes squirrels, hares, and mole rats, though they will also eat lizards, snakes, frogs and birds. When a serval is hunting, it prowls slowly through grasslands, pausing for as long as 15 minutes at a time to listen for prey. When they find something, they leap high with all four feet off the ground, then pounce on their victim, stunning it with a blow from their forefeet. If they miss, they will repeat the process (at the risk of looking somewhat like a wind-up toy). They don’t miss very often, though. One of every two pounces by a serval results in the capture of prey – making it one of the most effective feline hunters.
A wild cat that I particularly like is called the Fishing Cat. Fishing cats are native to Indochina, Pakistan, India, and southeast Asia, including the islands of Java and Sumatra. They live in densely vegetated areas near water, such as marshes, mangroves, rivers and streams. This is a fairly small cat, weighing 15-25 pounds and measuring 2 to 3 feet long, with short tails.
Their legs are very short and their heads very broad, giving them a very stocky appearance. They tend to be olive-gray in color, and have dark spots roughly arranged in stripes along their bodies. Fishing cats, as you might have guessed, eat mostly fish. Because of that, they do not have the same dread of water that most cats do – in fact, they dive into water to catch them! A fishing cat will tap their paws on the surface of the water, mimicking insect movement. When a fish comes close, the fishing cat will then dive in after it. They will also eat other aquatic animals, like frogs, snakes, and crustaceans. When a fishing cat is swimming, it can use its short tail like a rudder, helping it turn suddenly to catch their prey. In addition, their webbed paws can act like oars, or also give them extra traction in the mud. They are probably one of the few cats I have seen who don’t look completely bedraggled when wet!
The final wild cat of the day is the Margay. Margays are also known as tree ocelots or long tailed spotted
cats, and are native to Central and South America. Their fur is patterned much like an ocelot: a yellow-brown body covered with black spots and whorls, as well as white underbellies. They are one of the smallest cats in the cat family, only weighing 4-6 pounds and measuring 1.5 to 2.5 feet long (with an extra foot for the tails). These are also the most accomplished climbers of all cats, too. Their light bodies and strong paws help in that, as do their specially adapted claws and ankle joints. These can rotate 180 degrees, which allows them to move almost like monkeys through tree branches – including running down trunks head first, crawling along the underside of branches, or hanging from a branch with 1 leg!
I think it’s fun to compare traits of wild cats with my 2 housecats. Like caracals and servals, both of my cats like to stalk their “prey” (though, since they are indoor cats, their prey consists mostly of catnip-stuffed mice). They have also been known to pounce with a wild, all-4-feet-in-the-air jump. However, unlike fishing cats, they absolutely hate being wet (baths are a particularly unpleasant time for them). And they are nowhere near as coordinated as a margay. In fact, they have been known to fall off the couch from time to time, so I definitely would not trust them in a tree!
The pictures I posted here are from the following websites:
Caracal: http://wildfeline.tripod.com/african_cats.htm
Serval: http://www.vulkaner.no/n/africa/somaliwildlife-n.html
Fishing Cat: http://www.bigcatrescue.org/fishing_cat_photos.htm
Margay: http://www.guyana.org/Guyana_Photo_Gallery/animals/animals2.html
The list of wild cats is vast and varied. You probably already know about the most famous of the wild cats, such as lions, tigers, cheetahs and leopards. But I thought I’d take some time to tell you about a few wild cats that you might not have heard of before.
For example, take the caracal. The caracal, also known as the African Lynx or the Deser
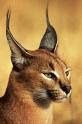
Another relatively obscure wild cat is the serval. Servals are considered medium-sized wild cats; though their weight is comparable with the caracal (20-45 pounds), their bodies are significantly longer (3 feet in length, plus a foot and a half for the tail). This makes them very slender animals, an image assisted by the fact that they have one of the longest legs in the cat family. (
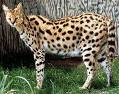
A wild cat that I particularly like is called the Fishing Cat. Fishing cats are native to Indochina, Pakistan, India, and southeast Asia, including the islands of Java and Sumatra. They live in densely vegetated areas near water, such as marshes, mangroves, rivers and streams. This is a fairly small cat, weighing 15-25 pounds and measuring 2 to 3 feet long, with short tails.
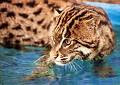
The final wild cat of the day is the Margay. Margays are also known as tree ocelots or long tailed spotted
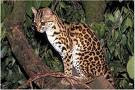
I think it’s fun to compare traits of wild cats with my 2 housecats. Like caracals and servals, both of my cats like to stalk their “prey” (though, since they are indoor cats, their prey consists mostly of catnip-stuffed mice). They have also been known to pounce with a wild, all-4-feet-in-the-air jump. However, unlike fishing cats, they absolutely hate being wet (baths are a particularly unpleasant time for them). And they are nowhere near as coordinated as a margay. In fact, they have been known to fall off the couch from time to time, so I definitely would not trust them in a tree!
The pictures I posted here are from the following websites:
Caracal: http://wildfeline.tripod.com/african_cats.htm
Serval: http://www.vulkaner.no/n/africa/somaliwildlife-n.html
Fishing Cat: http://www.bigcatrescue.org/fishing_cat_photos.htm
Margay: http://www.guyana.org/Guyana_Photo_Gallery/animals/animals2.html
Monday, November 5, 2007
Now that’s an old clam!
Well, I’ve written about animals that live a long time, and I’ve written about clams. So I guess now would be a good time for an entry on clams that live a really long time!
Scientists at Bangor University in the United Kingdom have found a quahog clam that holds the title of oldest animal in the world – at a whopping 405 years. Now that’s old.
So what are quahogs? Quahogs (pronounced “KO-hogs”) are hard shell clams. If you picture a clam on the menu in a seafood restaurant, you’re thinking of a quahog. They, along with soft-shell clams, oysters, scallops and mussels, are known as bivalve mollusks. That’s because their shelves are made of two halves, or valves. The valves connect at a joint called the hinge, which contains the oldest part of the clam’s shell. That part is known as the beak. The hinge will be in an open position and the valves ajar if possible, but if water conditions are bad or there are predators around, the clam can snap its valves shut, and hold itself tightly closed for as long as necessary.
Quahogs have several muscles that are important for their physiology. Their adductor muscles control the opening and closing of the hinge. They also have a foot for burrowing in the sand, which is controlled by the foot retractor muscles. One other important feature of their bodies is their necks. A quahog’s neck can stick upwards through the sediment and into the water above. Through the incurrent siphons in their necks, they filter water over their gills, collecting algae, plankton and diatoms to eat. The filtered water then gets spit back out through their excurrent siphons.
There are actually 2 different species of quahogs in the world. The clam that we eat in North America is called Mercenaria mercenaria. These clams are also known as Northern quahogs, round clams, chowder clams, littlenecks, topnecks and cherrystones. You might wonder at their scientific name, which is related to the Latin word for money (“mercenaria” means “something of value”). Apparently, Native American tribes in New England used to use quahog shells to make valuable beads for barter. In addition to the Northern quahog, however, there is another species native to the North Atlantic ocean. This species is called Arctica islandica, and it is this variety of quahog that was found to be over 400 years old. It was collected from water over 250 feet deep off the coast of Iceland, and it beats the previous record holder for longest-lived clam by several decades.
So how do the scientists know how old these clams are? Remember, the oldest part of the clam’s shell is the beak, which is found near the hinge. Every year a quahog is alive, its shell grows outwards from its beak. And every year, the growth creates a ring in the shell. Scientists can simply count the number of rings to see how many years of growth the shell has undergone. This is very similar to the way that trees are dated.
How do these clams live so long? No one really knows, though the finders of this old quahog speculate that it may be due to slow cell division. Scientists think that geoducks, another kind of clam, can live 100+ years because they have a low-stress lifestyle (you can read my entry “What do you call that?” if you missed it before). So an alternate theory could be that perhaps quahogs in the waters off Iceland live even easier lives than geoducks in the Puget Sound!
I don’t know if the same could be said for the North American quahog, though. There is a large and active clamming industry in American and Canada, which harvests quahogs for consumption around the world. So it’s a safe bet that they do not live as long as their Icelandic cousins.
You can find an article describing this clam on National Geographic Online:
http://news.nationalgeographic.com/news/2007/10/071029-oldest-clam.html.
Scientists at Bangor University in the United Kingdom have found a quahog clam that holds the title of oldest animal in the world – at a whopping 405 years. Now that’s old.
So what are quahogs? Quahogs (pronounced “KO-hogs”) are hard shell clams. If you picture a clam on the menu in a seafood restaurant, you’re thinking of a quahog. They, along with soft-shell clams, oysters, scallops and mussels, are known as bivalve mollusks. That’s because their shelves are made of two halves, or valves. The valves connect at a joint called the hinge, which contains the oldest part of the clam’s shell. That part is known as the beak. The hinge will be in an open position and the valves ajar if possible, but if water conditions are bad or there are predators around, the clam can snap its valves shut, and hold itself tightly closed for as long as necessary.
Quahogs have several muscles that are important for their physiology. Their adductor muscles control the opening and closing of the hinge. They also have a foot for burrowing in the sand, which is controlled by the foot retractor muscles. One other important feature of their bodies is their necks. A quahog’s neck can stick upwards through the sediment and into the water above. Through the incurrent siphons in their necks, they filter water over their gills, collecting algae, plankton and diatoms to eat. The filtered water then gets spit back out through their excurrent siphons.
There are actually 2 different species of quahogs in the world. The clam that we eat in North America is called Mercenaria mercenaria. These clams are also known as Northern quahogs, round clams, chowder clams, littlenecks, topnecks and cherrystones. You might wonder at their scientific name, which is related to the Latin word for money (“mercenaria” means “something of value”). Apparently, Native American tribes in New England used to use quahog shells to make valuable beads for barter. In addition to the Northern quahog, however, there is another species native to the North Atlantic ocean. This species is called Arctica islandica, and it is this variety of quahog that was found to be over 400 years old. It was collected from water over 250 feet deep off the coast of Iceland, and it beats the previous record holder for longest-lived clam by several decades.
So how do the scientists know how old these clams are? Remember, the oldest part of the clam’s shell is the beak, which is found near the hinge. Every year a quahog is alive, its shell grows outwards from its beak. And every year, the growth creates a ring in the shell. Scientists can simply count the number of rings to see how many years of growth the shell has undergone. This is very similar to the way that trees are dated.
How do these clams live so long? No one really knows, though the finders of this old quahog speculate that it may be due to slow cell division. Scientists think that geoducks, another kind of clam, can live 100+ years because they have a low-stress lifestyle (you can read my entry “What do you call that?” if you missed it before). So an alternate theory could be that perhaps quahogs in the waters off Iceland live even easier lives than geoducks in the Puget Sound!
I don’t know if the same could be said for the North American quahog, though. There is a large and active clamming industry in American and Canada, which harvests quahogs for consumption around the world. So it’s a safe bet that they do not live as long as their Icelandic cousins.
You can find an article describing this clam on National Geographic Online:
http://news.nationalgeographic.com/news/2007/10/071029-oldest-clam.html.
Friday, November 2, 2007
A Medical Use for Capsaicin
A few weeks ago, I wrote about a molecule called capsaicin, which is the ingredient that makes peppers spicy (‘Those red hot chili peppers”). Apparently, capsaicin is not only used in cooking – it also has medicinal value. Scientists are testing whether capsaicin can be used as a painkiller.
This may sound a bit odd. After all, capsaicin causes pain, doesn’t it? (Try pouring some tabasco sauce straight into your mouth and see how you feel.) It does, but here’s the catch. If you apply enough capsaicin to a nerve cell, it will stimulate so much pain that the nerve will become numb. And numb nerves can no longer signal pain. So a brief pain associated with the capsaicin treatment can result in a long time of no pain at all.
This idea is being used to treat long-term or throbbing pain, which is caused by a very specific set of nerve endings. Called C fibers, they are a type of sensory fiber associated with chronic pain and warmth. C fibers possess a protein that bridges the membrane of the nerve ending. This protein, called TRPV1, acts like a gate, which is usually closed. However, when capsaicin comes along, it binds TRPV1 and opens the gate. As a result, calcium ions flood the cell, and if there’s enough calcium ions, the nerve goes numb. A high enough dose of capsaicin can result in numbness that can last several weeks, in fact.
Capsaicin works only on the C fiber nerve endings, not those that work in other kinds of pain or for movement. That means that numbness associated with capsaicin treatment would only block this pain signal. It would not interfere with any other kind of nerve process. If you’ve ever had your mouth numbed by the dentist, you may be able to appreciate the distinction. When you are treated with Novocaine, all the nerves in your mouth go numb, including those for sensation and movement. That’s why you lose feeling across your entire mouth (and perhaps your tongue and nose). A capsaicin-based anesthesia would be much more selective in its ability to block pain. So a dental filling would not only be pain-free, you would also be free of that awkward drooling that results from an immobile mouth.
Doctors are using this treatment in patients undergoing extremely painful surgery. They treat the nerves exposed during the operation with an ultra-pure form of capsaicin - since the patients are anesthetized, it doesn’t hurt any more than the surgery does. However, once they recover from the anesthesia, doctors hope that their nerves will be numbed enough to allow for better, less painful recovery. And early indications suggest that it works as predicted. In small tests of those recovering from either open hernia repair or knee replacement, those who received the capsaicin treatment reported less pain than their untreated counterparts.
For an article discussing this work, you can check out:
http://www.baltimoresun.com/news/health/bal-te.peppers30oct30,0,1228065.story
This may sound a bit odd. After all, capsaicin causes pain, doesn’t it? (Try pouring some tabasco sauce straight into your mouth and see how you feel.) It does, but here’s the catch. If you apply enough capsaicin to a nerve cell, it will stimulate so much pain that the nerve will become numb. And numb nerves can no longer signal pain. So a brief pain associated with the capsaicin treatment can result in a long time of no pain at all.
This idea is being used to treat long-term or throbbing pain, which is caused by a very specific set of nerve endings. Called C fibers, they are a type of sensory fiber associated with chronic pain and warmth. C fibers possess a protein that bridges the membrane of the nerve ending. This protein, called TRPV1, acts like a gate, which is usually closed. However, when capsaicin comes along, it binds TRPV1 and opens the gate. As a result, calcium ions flood the cell, and if there’s enough calcium ions, the nerve goes numb. A high enough dose of capsaicin can result in numbness that can last several weeks, in fact.
Capsaicin works only on the C fiber nerve endings, not those that work in other kinds of pain or for movement. That means that numbness associated with capsaicin treatment would only block this pain signal. It would not interfere with any other kind of nerve process. If you’ve ever had your mouth numbed by the dentist, you may be able to appreciate the distinction. When you are treated with Novocaine, all the nerves in your mouth go numb, including those for sensation and movement. That’s why you lose feeling across your entire mouth (and perhaps your tongue and nose). A capsaicin-based anesthesia would be much more selective in its ability to block pain. So a dental filling would not only be pain-free, you would also be free of that awkward drooling that results from an immobile mouth.
Doctors are using this treatment in patients undergoing extremely painful surgery. They treat the nerves exposed during the operation with an ultra-pure form of capsaicin - since the patients are anesthetized, it doesn’t hurt any more than the surgery does. However, once they recover from the anesthesia, doctors hope that their nerves will be numbed enough to allow for better, less painful recovery. And early indications suggest that it works as predicted. In small tests of those recovering from either open hernia repair or knee replacement, those who received the capsaicin treatment reported less pain than their untreated counterparts.
For an article discussing this work, you can check out:
http://www.baltimoresun.com/news/health/bal-te.peppers30oct30,0,1228065.story
Subscribe to:
Posts (Atom)