I’ve written a fair amount about sleep in the past. But there’s one thing that I haven’t yet written about as far as sleep is concerned – dreams. I dream a lot. Most mornings, I wake up with a memory of at least 1 of my dreams, if not pieces of many of them. It is an integral part of sleeping, therefore it is probably pretty important. I started looking into how and why we dream to answer a few of my questions about what makes it so important.
Let’s first deal with how we dream. Dreams occur during REM sleep, the part of your sleep cycle when your body is inactive and your brain is active. (To refresh your memory on the sleep cycle, you can read my entry “Zzzzz…” from September 21.) Dreaming seems to be based in very specific regions of your brain. Using a variety of technologies, scientists have found that the limbic system is the most active part of your brain during REM sleep.
The limbic system is a set of brain structures deep within your brain that has a broad effect. Overall, it regulates both the endocrine system and the autonomic nervous system. The endocrine system is responsible for the production of all the hormones in your body, and regulates metabolism, growth and development, tissue function and emotions. The autonomic nervous system is a master control system for your body, regulating body temperature, heart beat, digestion, breathing, and pupil dilation. The limbic system contains numerous brain structures to help it do all of these things, including the hippocampus (which regulates long-term memories), hypothalamus (which affects heart rate, blood pressure, thirst, hunger and the sleep/wake cycle), thalamus (which communicates with the cerebral cortex) and amygdala (which is involved in motivational stimuli such as fear and pleasure). It is also closely connected to the nucleus accumbens, which is the brain’s pleasure center.
During REM sleep, our brains are active. So why don’t our bodies respond by moving, like when we are awake? That’s because REM sleep involves not only the activation of the limbic system, but also the inactivation of the cells in the brain that control muscles. REM sleep also inactivates the part of the brain that handles judgment. This happens in the frontal lobe, one of the 4 hemispheres of the cerebrum. Inactivation of this judgment process means that we accept the bizarre and illogical features of our dreams as they happen, and easily forget them upon waking.
Okay, so we know at least a little of how we dream. Our brains activate the limbic system and inactivate our muscles and our judgment. But why do we do it? While there is no clear-cut answer, scientists have many different theories. Because the limbic system is involved in both sensory processing and emotions, many people have suggested that dreaming is a way for us to connect our emotions and our thoughts. This is the psychological school of thought, in which dreams are believed to help us deal with emotions and complicated thoughts that we can’t handle while conscious. Some, however, believe that there is a physiological basis for dreaming. This argument says that dreaming exercises the synapses in our brains while we are resting. When we are awake, our brains are processing billions of messages through our brain cells. Dreams would allow this continual flow of messages, even as our bodies and brains get the benefit of being asleep.
So let’s review. How do we dream? The answer lies in the regions of our brains that are active and inactive during REM sleep. Why do we dream? Who knows.
On a final note, here’s one interesting fact that I cam across in my research for this entry –the percent of our sleep that allows for dreaming decreases as we get older. Babies spend around 16 hours a day sleeping, and half of that is REM sleep. That’s 480 minutes a day. Adults (those over 50, anyways) only sleep around 6 hours per day. And only 15% of that time is spent in REM sleep. That’s only 54 minutes. What a difference age makes!
Friday, December 21, 2007
Monday, December 17, 2007
Funny things about humans
There are a number of funny things about our bodies that make me curious. So I have decided to devote an entry to a few miscellaneous things about the human anatomy that particularly intrigue me. For example, have you ever thought about why we have kneecaps? Or wondered why we have clumps of junk in the corners of our eyes when we wake up? Or how about earwax - what is that for?
First in my list of funny things about humans are kneecaps, also known as patella. The patella is a thick, triangular piece of bone that sits on top of your knee, covering and protecting it. It’s 1 of 4 bones in your knee, including the tibia (shin bone), the fibula (parallel to the tib
ia) and the femur (thigh bone). The kneecap is connected to the rest of your knee through tendons, ligaments and cartilage. The quadriceps tendon connects it to your thigh muscles, multiple ligaments connect it to the bones of the lower leg, and cartilage surrounds the whole thing. The patella is a sesamoid bone, which means it is a bone embedded within a tendon. (Do you remember my entry on the funny bone? The patellar tendon is the one that gets tapped in a knee jerk reflex test.) Sesamoid bones function to increase the mechanical effect of a particular tendon; that’s why they are mostly found in the hands, feet and knees. So the kneecap increases the leverage of the knee joint – actually, it increases the extension strength of your knee by about 30%.
Knees are complicated joints, and they have many things that can go wrong. There are several problems associated with kneecaps. Runner’s knee (or Chondrolmalacia) occurs when the cartilage directly underneath the kneecap becomes irritated. This is because the patella is directly rubbing against one side of the knee joint. Another problem is housemaid’s knee (or Prepatellar bursitis). There is a small lubricating sac between your skin and the surface of your kneecap called the bursa. People who spend a lot of time on their knees often have their patellar bursa become inflamed, causing swelling over the knee and limiting movement. Still another common problem is patellar dislocation. Your kneecap slides in a grove along your thigh bone as your knee extends. In some people, the patella moves out of that groove, and becomes dislocated. Kneecap dislocation is a fairly painful problem, underscoring how important it is for our knees for our kneecaps to be positioned properly. All in all, I’d say that kneecaps are really an underappreciated piece of our bodies.
Now on to another funny piece of our anatomies – eye gunk. Perhaps you call it crusts, sleepy eyes, or (here’s a truly unscientific name) eye goobers. Whatever it’s name, I’m talking about the clumps that accumulate in the corners of our eyes when we wake up in the morning. This junk is what’s left over from our tears once the water has gone away. Tears have 3 components – salt water, protein and fat. The salt water is produced by the tear gland, which sits behind the upper outer corner of the eyes. Protein is secreted by the conjunctiva, the clear film over the surface of the eye. And the fat comes from ducts in the eyelids. All 3 of these things serve very important functions. They clean the eye, help correct for imperfections in the cornea, and deliver nutrients to the eye. When you sleep, however, most of these things aren’t needed. We don’t get dirt in our eyes and we don’t need have the imperfections in the cornea corrected because we’re not looking at anything. So we don’t make tears. The liquid already on the surface of your eye seeps out as you sleep, and the water dries up. This leaves behind clumps of fat and protein. We don’t get this when we’re awake, because our eyes are always producing new liquid. So, tomorrow morning, if you find crusts in your eyes, you can tell yourself – look, it’s protein and fat!
Today’s final topic is earwax. Earwax is more properly known as cerumen (pronounced suh-ROO-mun). It is a yellow, waxy substance secreted by glands in the ear canal of many mammals, including humans. In humans, 2 types of glands in the outer ear canal produce the wax – the ceruminous glands (which are modified sweat glands) and sebaceous glands. It’s made up of over 40 different components, including wax and oils. The most abundant component is keratin, an extremely important protein found in all the outermost layers of our skin. Everyone’s earwax is unique, with a specific composition, color and consistency. Color can range from golden yellow to black – though no one knows what pigment is responsible for this coloration. Consistency can be either dry or wet – and that seems to be genetically determined, as dry earwax is common in Asians and Native Americans, while wet earwax is common in African-Americans and Caucasians.
Earwax is actually very important for your ears. It protects and moisturizes the inner ear canal. It helps prevent both bacterial and fungal infections within the ear canal. It keeps dirt, dust, and other unsavory things from accessing the inner portions of your ears. And it helps move dead skin cells out from the inside of the ear to be shed away. On your skin, dead cells are sloughed off through friction. Within the ear canal, the movement of your ears through talking, chewing and swallowing cause the earwax to rub against the cells, picking them up. Small hairs called cilia then move the earwax and dead cells towards the outside just like a conveyor belt. Without this, we would have no way of shedding dead cells from within our ear canals. All in all, while this is certainly something I’ve never really fully appreciated before, we should really be grateful for our earwax!
These are just a few of the intriguing questions I have about the human body. I have many others – but I’ll have to save those for another time. How about you? What about the human body makes you sit back and think, “Why on earth do we have that?”
The image of the kneecap was taken from:
http://orthopedics.about.com/cs/patelladisorders/a/kneecap.htm
First in my list of funny things about humans are kneecaps, also known as patella. The patella is a thick, triangular piece of bone that sits on top of your knee, covering and protecting it. It’s 1 of 4 bones in your knee, including the tibia (shin bone), the fibula (parallel to the tib
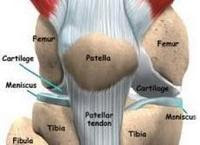
Knees are complicated joints, and they have many things that can go wrong. There are several problems associated with kneecaps. Runner’s knee (or Chondrolmalacia) occurs when the cartilage directly underneath the kneecap becomes irritated. This is because the patella is directly rubbing against one side of the knee joint. Another problem is housemaid’s knee (or Prepatellar bursitis). There is a small lubricating sac between your skin and the surface of your kneecap called the bursa. People who spend a lot of time on their knees often have their patellar bursa become inflamed, causing swelling over the knee and limiting movement. Still another common problem is patellar dislocation. Your kneecap slides in a grove along your thigh bone as your knee extends. In some people, the patella moves out of that groove, and becomes dislocated. Kneecap dislocation is a fairly painful problem, underscoring how important it is for our knees for our kneecaps to be positioned properly. All in all, I’d say that kneecaps are really an underappreciated piece of our bodies.
Now on to another funny piece of our anatomies – eye gunk. Perhaps you call it crusts, sleepy eyes, or (here’s a truly unscientific name) eye goobers. Whatever it’s name, I’m talking about the clumps that accumulate in the corners of our eyes when we wake up in the morning. This junk is what’s left over from our tears once the water has gone away. Tears have 3 components – salt water, protein and fat. The salt water is produced by the tear gland, which sits behind the upper outer corner of the eyes. Protein is secreted by the conjunctiva, the clear film over the surface of the eye. And the fat comes from ducts in the eyelids. All 3 of these things serve very important functions. They clean the eye, help correct for imperfections in the cornea, and deliver nutrients to the eye. When you sleep, however, most of these things aren’t needed. We don’t get dirt in our eyes and we don’t need have the imperfections in the cornea corrected because we’re not looking at anything. So we don’t make tears. The liquid already on the surface of your eye seeps out as you sleep, and the water dries up. This leaves behind clumps of fat and protein. We don’t get this when we’re awake, because our eyes are always producing new liquid. So, tomorrow morning, if you find crusts in your eyes, you can tell yourself – look, it’s protein and fat!
Today’s final topic is earwax. Earwax is more properly known as cerumen (pronounced suh-ROO-mun). It is a yellow, waxy substance secreted by glands in the ear canal of many mammals, including humans. In humans, 2 types of glands in the outer ear canal produce the wax – the ceruminous glands (which are modified sweat glands) and sebaceous glands. It’s made up of over 40 different components, including wax and oils. The most abundant component is keratin, an extremely important protein found in all the outermost layers of our skin. Everyone’s earwax is unique, with a specific composition, color and consistency. Color can range from golden yellow to black – though no one knows what pigment is responsible for this coloration. Consistency can be either dry or wet – and that seems to be genetically determined, as dry earwax is common in Asians and Native Americans, while wet earwax is common in African-Americans and Caucasians.
Earwax is actually very important for your ears. It protects and moisturizes the inner ear canal. It helps prevent both bacterial and fungal infections within the ear canal. It keeps dirt, dust, and other unsavory things from accessing the inner portions of your ears. And it helps move dead skin cells out from the inside of the ear to be shed away. On your skin, dead cells are sloughed off through friction. Within the ear canal, the movement of your ears through talking, chewing and swallowing cause the earwax to rub against the cells, picking them up. Small hairs called cilia then move the earwax and dead cells towards the outside just like a conveyor belt. Without this, we would have no way of shedding dead cells from within our ear canals. All in all, while this is certainly something I’ve never really fully appreciated before, we should really be grateful for our earwax!
These are just a few of the intriguing questions I have about the human body. I have many others – but I’ll have to save those for another time. How about you? What about the human body makes you sit back and think, “Why on earth do we have that?”
The image of the kneecap was taken from:
http://orthopedics.about.com/cs/patelladisorders/a/kneecap.htm
Thursday, December 13, 2007
Purrrrrr…..
I love watching my 2 cats, especially when my older cat decides to sneak up on the younger one. She crouches down, her ears held low, the tip of her tail twitching. She stealthily prowls over (hiding behind the furniture, of course), until she is close enough. Then – pounce! – out she leaps. (Of course, my younger cat is very used to this game, so she isn’t often caught unawares!) She also does this to stalk the occasional insect that makes its way into the house. From everything that I’ve seen, a wild cat stalking its prey acts in a very similar fashion. With ears down and crouched low, they slink closer to the target, tail twitching - then they attack.
I love to think about ways in which domesticated cats are like their wild cousins. Stalking prey, cleaning themselves with their tongues, perching in high places to look over their territory, roughhousing with each other – wild and tame cats all do these things. But there’s one thing that tame cats do that I was unfamiliar with in their wild counterparts – purring. My cats purr a lot. But do wild cats do the same? Do lions purr on the African savannah, or tigers when they are relaxing in the jungle? For that matter, what is purring, anyways? And why do housecats do it?
Many people who’ve spent time around cats know that they purr when they are content, such as when they are cuddling on the lap of their favorite person. However, this isn’t the only time that they purr. It also happens when a mother cat is giving birth, when kittens are nursing, or when a cat is severely frightened or injured; cats will even purr as they are dying. An obvious question, therefore, is why?
It is possible that purring serves a mental purpose in cats. For example, a mother cat could be trying to soothe her newborn kittens. The kittens could telling their mother that they are okay. A gravely injured cat could be trying to comfort itself. A stressed cat could be trying to keep itself calm. It is also likely, however, that purring also serves a physiological role, as well. Scientists have shown that the measurable frequency range of a cat’s purr is consistently between 25 and 150 Hertz. This range of sound has been demonstrated to promote physical health, including bone growth and repair, pain relief and decreased inflammation. Apparently there used to be an old adage often repeated in veterinary school – “If you put a cat and a bunch of broken bones in the same room, the bones will heal.” It is highly likely that cats actually purr to help themselves get better – consistent with the observation of purring by injured or dying cats. In fact, here’s something very interesting – comparing cats and dogs, cats heal from injuries such as broken bones or even surgery better, faster, and with fewer complications than dogs.
So that’s what purring does. But how do cats do it? Actually, no one knows for sure. It could be from the hyoid bone, a small flexible bone in the neck. When a cat pushes air through its voicebox, the bone can rattle, possible causing the distinctive rumble of a purr. Alternatively, purring could be caused by false vocal cords, located slightly behind the real vocal cords. Yet another possibility is that it is caused by something called the “neural oscillator” stimulating the vibration of the vocal cords themselves.
This confusion over what makes a cat purr actually makes it difficult to say whether big cats purr or not. I’ve come across multiple references to the purring ability (or lack thereof) of lions, tigers, cheetahs, leopards and jaguars. Here’s what I believe to be the most reliable information. According to a paper published in 2002 in the journal Mammal Review, cats in the Pantherinae subfamily (this includes lions, tigers, leopards and jaguars) can make some snuffling, purr-like sounds as they exhale, but it is not true purring. Only cats in the Felinae subfamily (that is, bobcats, cheetahs, Eurasian lynxes, and pumas) have the ability to truly purr. One possible explanation for this is that Pantherinae cats have a rigid hyoid bone that cannot vibrate as well as the hyoid bone in the Felinae cats.
Don’t feel badly for those lions, tigers, leopards and jaguars, though. The construction of their hyoid bones give them the distinct ability to let out a ferocious roar. That’s something that their purring cousins cannot do. So it seems that there are really 2 kinds of cats in the world – those that can purr, and those that can roar.
Personally, I’m glad my cats are the purring kind.
I love to think about ways in which domesticated cats are like their wild cousins. Stalking prey, cleaning themselves with their tongues, perching in high places to look over their territory, roughhousing with each other – wild and tame cats all do these things. But there’s one thing that tame cats do that I was unfamiliar with in their wild counterparts – purring. My cats purr a lot. But do wild cats do the same? Do lions purr on the African savannah, or tigers when they are relaxing in the jungle? For that matter, what is purring, anyways? And why do housecats do it?
Many people who’ve spent time around cats know that they purr when they are content, such as when they are cuddling on the lap of their favorite person. However, this isn’t the only time that they purr. It also happens when a mother cat is giving birth, when kittens are nursing, or when a cat is severely frightened or injured; cats will even purr as they are dying. An obvious question, therefore, is why?
It is possible that purring serves a mental purpose in cats. For example, a mother cat could be trying to soothe her newborn kittens. The kittens could telling their mother that they are okay. A gravely injured cat could be trying to comfort itself. A stressed cat could be trying to keep itself calm. It is also likely, however, that purring also serves a physiological role, as well. Scientists have shown that the measurable frequency range of a cat’s purr is consistently between 25 and 150 Hertz. This range of sound has been demonstrated to promote physical health, including bone growth and repair, pain relief and decreased inflammation. Apparently there used to be an old adage often repeated in veterinary school – “If you put a cat and a bunch of broken bones in the same room, the bones will heal.” It is highly likely that cats actually purr to help themselves get better – consistent with the observation of purring by injured or dying cats. In fact, here’s something very interesting – comparing cats and dogs, cats heal from injuries such as broken bones or even surgery better, faster, and with fewer complications than dogs.
So that’s what purring does. But how do cats do it? Actually, no one knows for sure. It could be from the hyoid bone, a small flexible bone in the neck. When a cat pushes air through its voicebox, the bone can rattle, possible causing the distinctive rumble of a purr. Alternatively, purring could be caused by false vocal cords, located slightly behind the real vocal cords. Yet another possibility is that it is caused by something called the “neural oscillator” stimulating the vibration of the vocal cords themselves.
This confusion over what makes a cat purr actually makes it difficult to say whether big cats purr or not. I’ve come across multiple references to the purring ability (or lack thereof) of lions, tigers, cheetahs, leopards and jaguars. Here’s what I believe to be the most reliable information. According to a paper published in 2002 in the journal Mammal Review, cats in the Pantherinae subfamily (this includes lions, tigers, leopards and jaguars) can make some snuffling, purr-like sounds as they exhale, but it is not true purring. Only cats in the Felinae subfamily (that is, bobcats, cheetahs, Eurasian lynxes, and pumas) have the ability to truly purr. One possible explanation for this is that Pantherinae cats have a rigid hyoid bone that cannot vibrate as well as the hyoid bone in the Felinae cats.
Don’t feel badly for those lions, tigers, leopards and jaguars, though. The construction of their hyoid bones give them the distinct ability to let out a ferocious roar. That’s something that their purring cousins cannot do. So it seems that there are really 2 kinds of cats in the world – those that can purr, and those that can roar.
Personally, I’m glad my cats are the purring kind.
Monday, December 10, 2007
That’s not very funny!
Today’s topic is one that, if you have an elbow, I’m sure you are familiar with. I’m confident that, at some point, you’ve managed to whack your elbow in such a way that pins and needles shot through your arm, funny tingling went through your hands, or just downright pain reverberated through your elbow. I’ve done it more times than I can count. And, of course, whenever I do, I know exactly what to blame.
My funny bone.
But hitting your funny bone isn’t really very funny, is it? In fact, it hurts! I want to know – what is the funny bone? Is it really a bone? Why do we have one? And why is it called the funny bone, anyways? Shouldn’t it be called the hurts-a-lot bone?
Actually, the funny bone is not really a bone. It’s a nerve – specifically, the ulnar nerve, which connects your fourth and fifth fingers to your nervous system and helps control the movement of your hands. The ulnar nerve goes from your hand to your shoulder, passing around the back of your elbow. There, it sits directly on top of the humerus, the bone of your upper arm. Because of its position on top of the bone, the only protection this nerve has is the skin of your elbow. It has no other fatty cushion to serve as a shield. In fact, the ulnar nerve is the only exposed nerve in the human body. All the rest of your nerves are deeply embedded in deep tissue, ligaments or muscles. But because this nerve has no such protection, it can come into direct contact with your humerus. So when you bang your elbow just wrong, the ulnar nerve bumps into the bone, and it sends pain signals through the nerve. Because this nerve is the main connector for your ring and pinkie fingers, in fact, the most common pain associated with hitting your funny bone is tingling in those fingers. Some lucky people, however, don’t feel any real pain when they hit their funny bones, just a funny tingling feeling. And that’s probably why it’s called the funny bone.
So why do we have this exposed nerve in our bodies? It seems like a strange place to put it, doesn’t it? Unfortunately, there isn’t a lot of choice when it comes to the nerves in our elbows. Elbows are, by nature, a lot of bone and not much else. To connect our forearms to our upper arms, the ulnar nerve has to run up the backs of our elbows. This is unlike the joint on our legs that is analogous to our elbows – the knees. Knees have a lot more connective tissue surrounding them than elbows, and thus the nerves are much better protected.
Speaking of comparing knees and funny bones – is the funny bone comparable at all to the reflex kick you have when the doctor taps your knees with that little hammer? Actually, not really. That kick reaction is called the knee jerk, and it is caused by an impact on a tendon. The patellar tendon runs down from your quadriceps muscles (in the front of your thigh), over your kneecap, and down to the lower leg. When the knee is held loosely at a 90 degree angle, with your lower leg hanging freely, and the patellar tendon is tapped, it sends a message to your nervous system that the tendon and the attached muscles have been stretched. The nervous system then sends a rapid message back to the muscle to contract, causing your thigh muscles to tighten. And this tightening makes your lower leg kick out.
So the funny bone and the knee jerk are 2 different physiological reactions. Personally, I much prefer the knee jerk reflex to hitting my funny bone. The knee jerk reaction is interesting. The funny bone reaction – well, I think it’s just painful.
My funny bone.
But hitting your funny bone isn’t really very funny, is it? In fact, it hurts! I want to know – what is the funny bone? Is it really a bone? Why do we have one? And why is it called the funny bone, anyways? Shouldn’t it be called the hurts-a-lot bone?
Actually, the funny bone is not really a bone. It’s a nerve – specifically, the ulnar nerve, which connects your fourth and fifth fingers to your nervous system and helps control the movement of your hands. The ulnar nerve goes from your hand to your shoulder, passing around the back of your elbow. There, it sits directly on top of the humerus, the bone of your upper arm. Because of its position on top of the bone, the only protection this nerve has is the skin of your elbow. It has no other fatty cushion to serve as a shield. In fact, the ulnar nerve is the only exposed nerve in the human body. All the rest of your nerves are deeply embedded in deep tissue, ligaments or muscles. But because this nerve has no such protection, it can come into direct contact with your humerus. So when you bang your elbow just wrong, the ulnar nerve bumps into the bone, and it sends pain signals through the nerve. Because this nerve is the main connector for your ring and pinkie fingers, in fact, the most common pain associated with hitting your funny bone is tingling in those fingers. Some lucky people, however, don’t feel any real pain when they hit their funny bones, just a funny tingling feeling. And that’s probably why it’s called the funny bone.
So why do we have this exposed nerve in our bodies? It seems like a strange place to put it, doesn’t it? Unfortunately, there isn’t a lot of choice when it comes to the nerves in our elbows. Elbows are, by nature, a lot of bone and not much else. To connect our forearms to our upper arms, the ulnar nerve has to run up the backs of our elbows. This is unlike the joint on our legs that is analogous to our elbows – the knees. Knees have a lot more connective tissue surrounding them than elbows, and thus the nerves are much better protected.
Speaking of comparing knees and funny bones – is the funny bone comparable at all to the reflex kick you have when the doctor taps your knees with that little hammer? Actually, not really. That kick reaction is called the knee jerk, and it is caused by an impact on a tendon. The patellar tendon runs down from your quadriceps muscles (in the front of your thigh), over your kneecap, and down to the lower leg. When the knee is held loosely at a 90 degree angle, with your lower leg hanging freely, and the patellar tendon is tapped, it sends a message to your nervous system that the tendon and the attached muscles have been stretched. The nervous system then sends a rapid message back to the muscle to contract, causing your thigh muscles to tighten. And this tightening makes your lower leg kick out.
So the funny bone and the knee jerk are 2 different physiological reactions. Personally, I much prefer the knee jerk reflex to hitting my funny bone. The knee jerk reaction is interesting. The funny bone reaction – well, I think it’s just painful.
Tuesday, December 4, 2007
Ayumu, the amazing chimpanzee
A well-written headline can really catch your eye. Like this one, from an article on National Geographic Online: “Young Chimp Outscores College Students in Memory Test.” I saw that and, of course, had to read the article!
This story is about a study done recently in Japan to test the short-term memory abilities of humans and chimpanzees, and published in the December volume of Current Biology. This test involved 3 chimpanzees (who know the numbers 1 through 9) and 12 human volunteers. The subjects were placed in front of a touch computer screen that displayed 9 numbers in various places around t
he screen. The task was to touch the numbers in numerical order from 1 to 9. Sounds pretty easy, right? Sure, until you add the last tricky part. Once the number 1 was pressed, the rest of the numbers were covered by white squares. The challenge, therefore, was to remember where each number had been, and still be able to touch them in the proper sequence. Of the subject tested, both the chimps and the humans were about the same in accuracy. But the chimps did it faster.
A second test was done, this time pitting the fastest chimp (named Ayumu) against 9 college students. This time, 5 numbers were flashed briefly on the screen, then covered up with white squares. Again, the subjects had to touch the numbers in order. When the subjects saw the numbers for a long period of time – almost 1 second – both humans and Ayumu performed equally well. However, when the numbers were shown only very briefly – 2 to 4 tenths of a second – Ayumu performed much better than the humans. He was correct approximately 80% of the time, while the human scores dropped by half, to 40%. Ayumu was much better at taking in the whole picture extremely rapidly, and remembering the positions of the numbers, than were his human competitors. I looked at the videos of Ayumu performing the test, and it was pretty incredible. The numbers would flash on the screen and then disappear so quickly that I could hardly even find the first one! But Ayumu consistently touched the numbers in the right order. I was really impressed.
Now, before we start bemoaning the poor intellectual abilities of the humans studied here, one very important caveat must be mentioned. Ayumu is only 5 years old. Chimpanzees can live to be 50 or 60 years old, so he’s still several years away from adulthood. The humans he competed with were in their early 20s, past the age of adolescence. The test uses the ability to handle "eidetic imagery," also known as photographic memory or total recall. This is the ability to remember complex spatial arrangements or sounds with extreme accuracy. Eidetic imagery is known to decrease with age; children perform much better on tests of eidatic imagery than do adults. Perhaps what was really shown in this test was eidatic imagery in chimpanzees? Quite possibly, actually, since Ayumu performed much better on the memory tests than did the older chimps in the study, including Ayumu’s mother, Ai. In fact, Ai actually performed worse on the tests than did the human subjects. So perhaps a better comparison to understand how good Ayumu’s memory is would be to compare him to a human 8-year-old.
The study in question was performed by researcher Tetsuro Matsuzawa of Kyoto University. Dr. Matsuzawa is a leading figure in the field of chimpanzee intelligence. Most of his work is known as the “Ai-project,” which studies the language and number skills of Ai (who is, if you remember, the mother of Ayumu). Ai is 29 years old, and is part of an established colony of chimpanzees in Kyoto, a group that has been studied for intelligence since 1978. That makes this paper one of many from the longest-running study on chimpanzee intelligence in the world.
This story is about a study done recently in Japan to test the short-term memory abilities of humans and chimpanzees, and published in the December volume of Current Biology. This test involved 3 chimpanzees (who know the numbers 1 through 9) and 12 human volunteers. The subjects were placed in front of a touch computer screen that displayed 9 numbers in various places around t
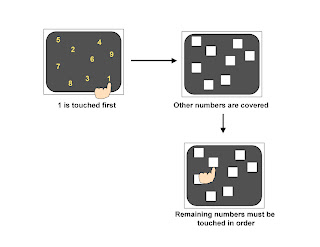
A second test was done, this time pitting the fastest chimp (named Ayumu) against 9 college students. This time, 5 numbers were flashed briefly on the screen, then covered up with white squares. Again, the subjects had to touch the numbers in order. When the subjects saw the numbers for a long period of time – almost 1 second – both humans and Ayumu performed equally well. However, when the numbers were shown only very briefly – 2 to 4 tenths of a second – Ayumu performed much better than the humans. He was correct approximately 80% of the time, while the human scores dropped by half, to 40%. Ayumu was much better at taking in the whole picture extremely rapidly, and remembering the positions of the numbers, than were his human competitors. I looked at the videos of Ayumu performing the test, and it was pretty incredible. The numbers would flash on the screen and then disappear so quickly that I could hardly even find the first one! But Ayumu consistently touched the numbers in the right order. I was really impressed.
Now, before we start bemoaning the poor intellectual abilities of the humans studied here, one very important caveat must be mentioned. Ayumu is only 5 years old. Chimpanzees can live to be 50 or 60 years old, so he’s still several years away from adulthood. The humans he competed with were in their early 20s, past the age of adolescence. The test uses the ability to handle "eidetic imagery," also known as photographic memory or total recall. This is the ability to remember complex spatial arrangements or sounds with extreme accuracy. Eidetic imagery is known to decrease with age; children perform much better on tests of eidatic imagery than do adults. Perhaps what was really shown in this test was eidatic imagery in chimpanzees? Quite possibly, actually, since Ayumu performed much better on the memory tests than did the older chimps in the study, including Ayumu’s mother, Ai. In fact, Ai actually performed worse on the tests than did the human subjects. So perhaps a better comparison to understand how good Ayumu’s memory is would be to compare him to a human 8-year-old.
The study in question was performed by researcher Tetsuro Matsuzawa of Kyoto University. Dr. Matsuzawa is a leading figure in the field of chimpanzee intelligence. Most of his work is known as the “Ai-project,” which studies the language and number skills of Ai (who is, if you remember, the mother of Ayumu). Ai is 29 years old, and is part of an established colony of chimpanzees in Kyoto, a group that has been studied for intelligence since 1978. That makes this paper one of many from the longest-running study on chimpanzee intelligence in the world.
Which way is up?
Let’s engage in a little thought experiment for a minute.
Imagine that you are a cell on the inner surface of your intestine. Your job is to transfer food (or rather, the nutrients resulting from food’s digestion) from the interior of the intestine to the bloodstream. It’s really important, therefore, that you know which side of you faces the intestine and which side faces the bloodstream. But how do you know which side is which?
Now imagine that you are a plant; specifically, you are a part of the plant that is about to bud into a leaf. It’s really important, therefore, that you develop as a leaf, and not as a flower or a root or an extension of the stem. But how do you know that which end of you is the part that connects back to the stem, and which part is supposed to flatten out into the leaf tip?
Finally, imagine that you are a developing fly. (Gross, perhaps, but bear with me.) As you go from embryo to larva, your body must pattern itself in such a way that you wind up with a head at one end, wings in the middle, and a tail at the other. It’s really important your body gets oriented correctly, or else you won’t hatch. But how do you know which end is supposed to be the head?
If you look at the world from a human perspective, it’s easy to know how to orient yourself for any task – can I walk on my feet, could I sit on a chair, or do I have to stand on my head? From the perspective of a cell, however, the task of orienting yourself is a little trickier. But it’s extremely important. If a cell gets its orientation wrong, the consequences could be dire – nutrients won’t get sent into the bloodstream, neurons will not send the right messages to the right places, entire pieces of anatomy could develop incorrectly, even entire organisms could die. This question of how cells orient themselves properly is quite a large topic. Every single cell in the world has “directionality” – top and bottom, left and right, front and back. How they do this is vast and complicated, and I’m not going to go into all of the ways it is done today. I just want to highlight a specific example: how does a developing fly know which end is the head and which is the tail?
A fly’s life begins with the fertilization of an egg. This single cell will then divide over and over to produce thousands of cells needed to make the final fly. And this single cell already contains the information to determine which end is the head and which end is the tail. The information is crucial from the very start of the fly’s life, because the early cells that are produced build on that information to reinforce the body plan. This all happens through the use of protein gradients.
Take a look at the diagram below. I’ve drawn an example of the protein gradients that help determine a fly’s head and tail. The single egg contains a gradient of proteins, which are called maternal-effect proteins. In this picture, there are 2 such proteins – blue and pink. There is a lot of blue at what will be the head, and a lot of pink at what will be the tail. In the middle, there is a mixture of both. Cell that are surrounded by a lot of blue protein know t
hat they are in the head, cells surrounded by a lot of pink protein know that they are in the tail, and cells with some of both know that they are somewhere in the middle. These maternal-effect proteins cause each cell to produce different proteins themselves, based on where they are. First come the gap proteins, which subdivide the body plan. The gap proteins then specify production of the pair-rule proteins, which make segments along the body. Finally, the pair-rule proteins specify which segment polarity proteins get made, which tell each segment which end points to the head and which to the tail. The combined action of all of these proteins tell each cell where it is in relation to the overall body. And that information helps each cell develop properly – so that the fly gets wings on his back, legs from his belly and eyes on his head.
This model of using protein gradients to specify orientation is commonly used in developing multicellular organisms, including mammals. Of course, as the animal gets more complex (with limbs, organs, and highly developed brains), the system of patterning gets more complex, too. It’s a topic that is being intensely studied among developmental biologists to this day. And it will probably continue to be studied for many years to come.
Imagine that you are a cell on the inner surface of your intestine. Your job is to transfer food (or rather, the nutrients resulting from food’s digestion) from the interior of the intestine to the bloodstream. It’s really important, therefore, that you know which side of you faces the intestine and which side faces the bloodstream. But how do you know which side is which?
Now imagine that you are a plant; specifically, you are a part of the plant that is about to bud into a leaf. It’s really important, therefore, that you develop as a leaf, and not as a flower or a root or an extension of the stem. But how do you know that which end of you is the part that connects back to the stem, and which part is supposed to flatten out into the leaf tip?
Finally, imagine that you are a developing fly. (Gross, perhaps, but bear with me.) As you go from embryo to larva, your body must pattern itself in such a way that you wind up with a head at one end, wings in the middle, and a tail at the other. It’s really important your body gets oriented correctly, or else you won’t hatch. But how do you know which end is supposed to be the head?
If you look at the world from a human perspective, it’s easy to know how to orient yourself for any task – can I walk on my feet, could I sit on a chair, or do I have to stand on my head? From the perspective of a cell, however, the task of orienting yourself is a little trickier. But it’s extremely important. If a cell gets its orientation wrong, the consequences could be dire – nutrients won’t get sent into the bloodstream, neurons will not send the right messages to the right places, entire pieces of anatomy could develop incorrectly, even entire organisms could die. This question of how cells orient themselves properly is quite a large topic. Every single cell in the world has “directionality” – top and bottom, left and right, front and back. How they do this is vast and complicated, and I’m not going to go into all of the ways it is done today. I just want to highlight a specific example: how does a developing fly know which end is the head and which is the tail?
A fly’s life begins with the fertilization of an egg. This single cell will then divide over and over to produce thousands of cells needed to make the final fly. And this single cell already contains the information to determine which end is the head and which end is the tail. The information is crucial from the very start of the fly’s life, because the early cells that are produced build on that information to reinforce the body plan. This all happens through the use of protein gradients.
Take a look at the diagram below. I’ve drawn an example of the protein gradients that help determine a fly’s head and tail. The single egg contains a gradient of proteins, which are called maternal-effect proteins. In this picture, there are 2 such proteins – blue and pink. There is a lot of blue at what will be the head, and a lot of pink at what will be the tail. In the middle, there is a mixture of both. Cell that are surrounded by a lot of blue protein know t
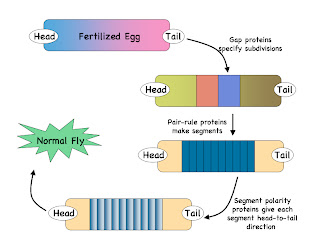
This model of using protein gradients to specify orientation is commonly used in developing multicellular organisms, including mammals. Of course, as the animal gets more complex (with limbs, organs, and highly developed brains), the system of patterning gets more complex, too. It’s a topic that is being intensely studied among developmental biologists to this day. And it will probably continue to be studied for many years to come.
Subscribe to:
Posts (Atom)